Recent research suggests that “blue” hydrogen might not be as beneficial for the climate as its proponents claim. But according to RFF’s Jay Bartlett and Alan Krupnick, blue hydrogen may be far greener with the right policy incentives.
Hydrogen is increasingly attracting attention from policymakers, investors, and industry leaders as a pathway to reduce greenhouse gas (GHG) emissions in sectors of the economy that are hard to decarbonize. In a report last year, we considered the potential for hydrogen to decrease emissions in the US industrial and power sectors and how a tax credit could support the production and use of decarbonized hydrogen.
Although the use of hydrogen does not result in GHG emissions, the current production of hydrogen—mainly through natural gas reforming (known as “gray” hydrogen, which predominates in the United States) or coal gasification (known as “brown” hydrogen, which predominates in China)—is very carbon intensive. One method of producing decarbonized hydrogen (so-called blue hydrogen) is to capture, and then utilize or store, the carbon dioxide (CO₂) emissions from gray or brown hydrogen. The other leading method for producing decarbonized hydrogen (so-called green hydrogen) is from splitting water using renewable or nuclear power.
Focus on the Life-Cycle Emissions of Blue Hydrogen
A new peer-reviewed study from Cornell University’s Robert Howarth and Stanford University’s Mark Z. Jacobson emphasizes that gray and blue hydrogen produce significant GHG emissions beyond the CO₂ generated at the hydrogen production plant. Most importantly, leaks along the natural gas supply chain allow some amount of methane to escape into the atmosphere. In addition, the production, processing, and transportation of natural gas generates some CO₂ emissions.
Because of these additional emissions and the high global warming potential of methane, the authors find that blue hydrogen may have only a modestly lower GHG footprint than gray hydrogen, and that using blue hydrogen for heat could be worse for the climate than burning natural gas or coal. Their findings—that blue hydrogen does not appear to be a significant means of decarbonization—have been publicized widely, including in the New York Times.
We appreciate the focus on the full life-cycle emissions of hydrogen, which is essential to designing hydrogen policies that have their intended effect on the climate. However, we find that the use of blue hydrogen can have a life-cycle climate impact that is both beneficial and substantial. Our conclusion differs from that of Howarth and Jacobson for two reasons: (i) the emissions of blue hydrogen should be compared to those of gray hydrogen, rather than to emissions from natural gas or coal, and (ii) given the technology and procurement options, blue hydrogen can have considerably lower life-cycle emissions than gray hydrogen.
What is the Emissions Benchmark for Blue Hydrogen?
A major factor driving the interest in decarbonized hydrogen is its versatility; hydrogen can be used in transportation, buildings, industry, and power. However, using decarbonized hydrogen as a source of heat—comparable to natural gas or coal—is challenged by other low-carbon options. For example, low-carbon heat for power generation or industrial processes can be provided by applying carbon capture, utilization, and storage (CCUS) directly to fossil fuel combustion, and zero-carbon heat for industrial processes or residential and commercial uses can come from renewable or nuclear power. With these alternatives, decarbonized hydrogen would need to be delivered at a low price per unit of energy to compete as a heat source in the United States, which would require a substantially reduced cost that may only be possible (eventually) for green hydrogen.
In contrast to the market for heat, the use of hydrogen as a feedstock in ammonia production and oil refining has no feasible near-term alternative. Decarbonized hydrogen thus is the only viable low-carbon pathway in these applications, and blue hydrogen is currently much less expensive than green hydrogen. Furthermore, the end products are identical for both the gray and blue hydrogen production processes; thus, blue hydrogen can substitute immediately for gray hydrogen. There is no need for new end-use equipment, such as hydrogen-burning turbines, which would be required for hydrogen to substitute for natural gas combustion in heating and power applications. Consequently, the near-term opportunity for blue hydrogen in the United States is likely to be concentrated in ammonia production and oil refining, so blue hydrogen is best compared with gray hydrogen when assessing life-cycle GHG emissions.
Blue Hydrogen Has the Potential for Large Reductions in Life-Cycle Emissions
Using gray hydrogen as a benchmark, we find that reductions in life-cycle emissions from blue hydrogen production can be significantly greater than Howarth and Jacobson estimate. In Figure 1, we present the component and total emissions of gray hydrogen and blue hydrogen, initially using the assumptions of Howarth and Jacobson and then using alternative assumptions that enable further emissions reductions. The component emissions include the following: (i) CO₂ from natural gas reforming and combustion (to produce heat for the process), (ii) methane leakage attributable to the natural gas supplied for reforming and process heat, (iii) CO₂ from the generation of electricity used to power carbon capture, (iv) methane leakage attributable to the natural gas used for electricity generation, and (v) upstream CO₂ emissions for the production, processing, and transportation of natural gas. We appreciate that Howarth and Jacobson have separated GHG emissions into these categories, which enables a transparent accounting of the components of blue hydrogen life-cycle emissions and indicates which process improvements or energy substitutions are needed to reduce or eliminate emissions within each component.
We present the analysis using a 20-year time frame in Figure 1a—which Howarth and Jacobson prefer, to emphasize the importance of reducing near-term GHG emissions—and the more conventional 100-year time frame (the primary time frame used by the US Environmental Protection Agency) in Figure 1b. Because methane is a more potent GHG than CO₂ but has a shorter half-life, a shorter time frame increases the impact of methane emissions on total GHG emissions.
Figure 1a. Life-Cycle Hydrogen Production Emissions, 20-Year Time Frame
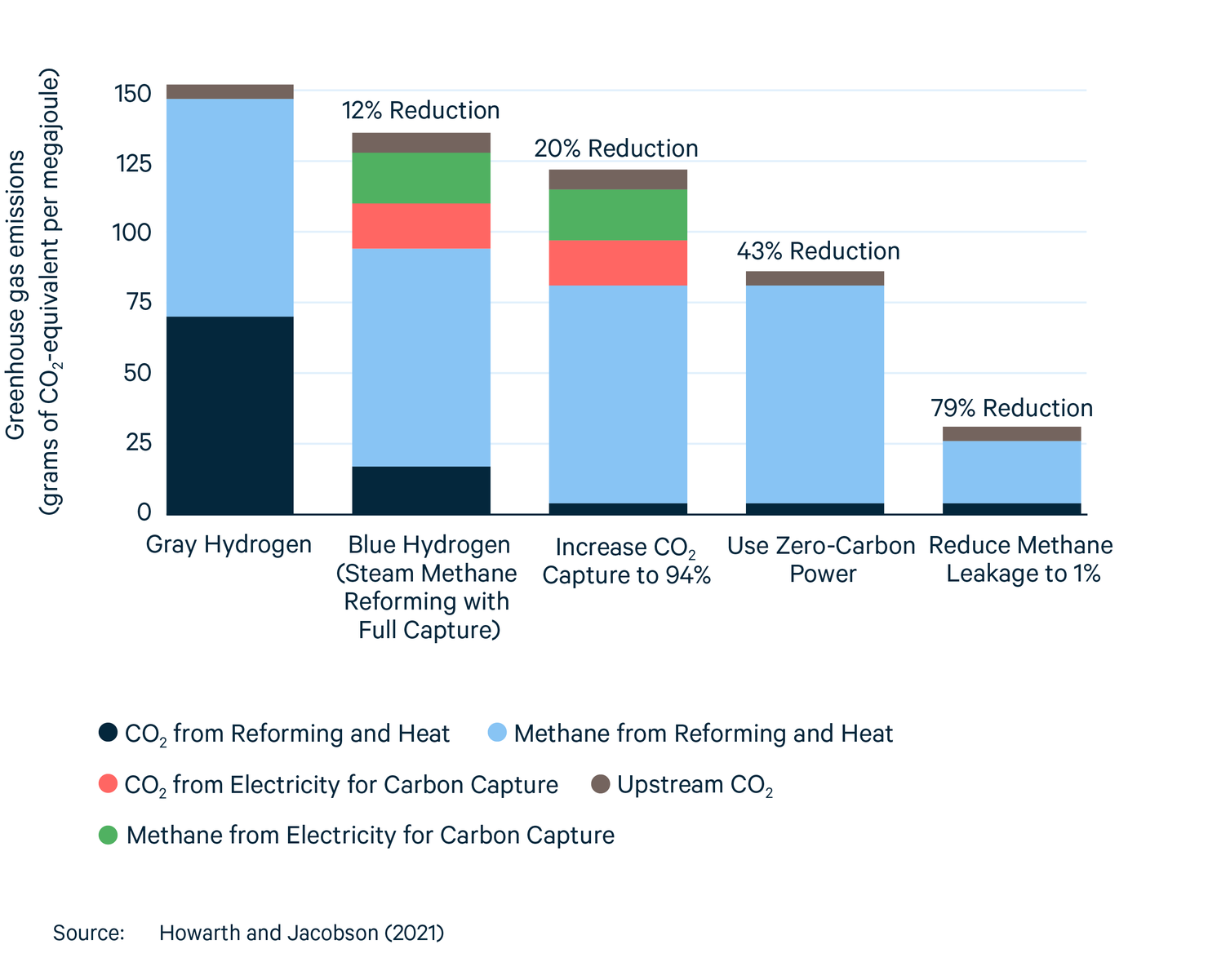
Figure 1b. Life-Cycle Hydrogen Production Emissions, 100-Year Time Frame
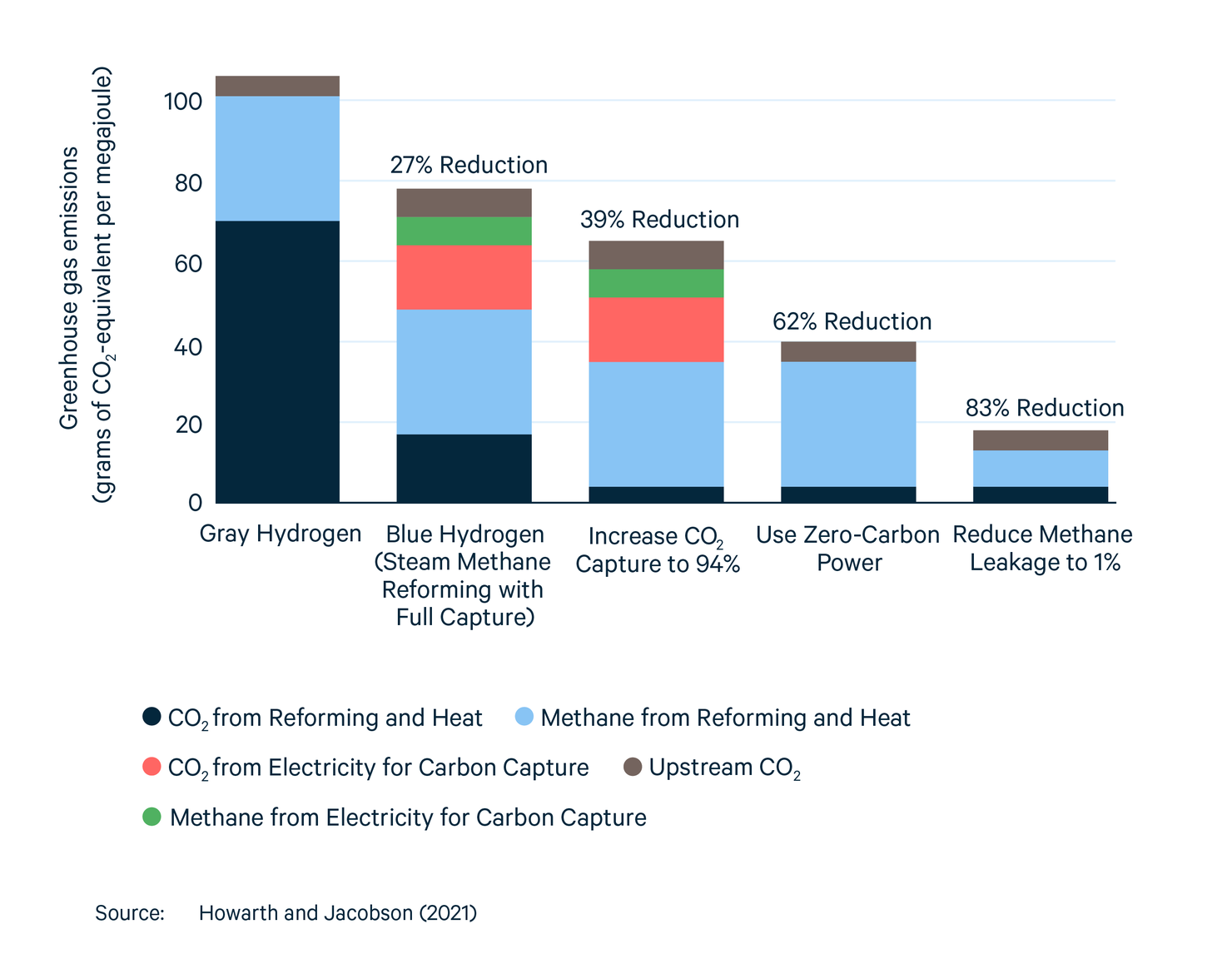
In the first and second columns of Figures 1a and 1b, we recreate the calculations of Howarth and Jacobson using their default assumptions. Gray hydrogen emissions (represented in Column 1) consist predominantly of the CO₂ generated on-site and the methane that has leaked along the natural gas supply chain. Column 2 shows the GHG emissions from an illustrative blue hydrogen facility that captures CO₂ from both the reforming process and the combustion exhaust. Specifically, the blue hydrogen facility is a steam methane reforming plant that captures 85 percent of the CO₂ in the concentrated process stream and 65 percent of the CO₂ in the dilute exhaust gases, resulting in the combined capture of 76 percent of the CO₂ generated on-site. However, CO₂ capture within the blue hydrogen facility does not reduce fugitive methane emissions from the natural gas used in reforming and combustion. Moreover, operating the CO₂ capture process consumes electricity, the generation of which causes direct CO₂ emissions, additional methane leakage, and a modest increase in upstream CO₂ emissions. In total, Howarth and Jacobson find that blue hydrogen reduces the GHG footprint of gray hydrogen by only 12 percent over a 20-year time frame and 27 percent over a 100-year time frame.
In Column 3, we increase the CO₂ capture rate from 76 percent to 94 percent to reflect a shift in the blue hydrogen production process, from steam methane reforming to an alternative technology: autothermal reforming. Unlike steam methane reforming, which emits CO₂ in the reforming process stream and the combustion exhaust, process and heating emissions are combined in autothermal reforming reactors, allowing for higher capture rates at lower cost. The massive H21 North of England project has proposed autothermal reforming reactors operating with a 94 percent CO₂ capture rate, and recent research finds that CO₂ capture rates over 95 percent are possible for autothermal reforming reactors using a novel separation technology. We should note that autothermal reforming with high rates of CO₂ capture may entail increased electricity consumption compared to steam methane reforming with lower CO₂ capture; we address power-related emissions in Column 4. [i]Howarth and Jacobson understandably use only steam methane reforming technology in their estimates, given that no blue hydrogen plants operating at scale currently use autothermal reforming technology. From projects under development and the latest research, we assume that autothermal reforming will allow for reduced emissions from future plants.
In Columns 4 and 5, we turn to the life-cycle GHG emissions from blue hydrogen beyond the CO₂ generated at the production plant. Column 4 illustrates the impact of running the CO₂ capture process with zero-carbon electricity (rather than gas-fired power), and Column 5 shows the effect of procuring low-emissions natural gas for reforming and heat (with a methane emissions rate of 1 percent versus the 3.5 percent rate assumed in Howarth and Jacobson). The 1 percent rate is more than possible. The 45 companies throughout the natural gas supply chain that belong to the industry group ONE Future Coalition aim to achieve a 1 percent methane emissions rate, or less, by 2025. Plus, the Oil and Gas Climate Initiative, which boasts membership by large international oil and gas companies and currently covers around 30 percent of global oil and gas production, has set a methane intensity target of 0.2 percent for all upstream emissions by 2025. In addition, trades of low-emissions natural gas are already taking place and certifications of low methane gas are taking off, fueled largely by demand from natural gas users, pressure from investors and company-rating agencies, and the growing activity of certification companies, such as MiQ. Regulatory pressure is also poised to grow, with Europe preparing regulations that will require low-emissions natural gas imports and Congress considering clean energy standard legislation giving credit to low-emissions natural gas, and various US states (such as New Mexico) taking action to regulate methane emissions.
Combining these three changes—a higher plant CO₂ capture rate, zero-carbon power, and low-emissions natural gas—the total reduction in life-cycle emissions for blue hydrogen relative to gray hydrogen is approximately 80 percent. Notably, this result holds whether we use a 20-year time frame or a 100-year time frame. In other words, blue hydrogen can have a significant climate benefit even over a short time horizon.
Appropriate Incentives for Blue Hydrogen Can Ensure a Beneficial Climate Effect
We are not claiming that blue hydrogen production necessarily will achieve such low emissions, and it is doubtful that such progress will be entirely realized without the requisite policy incentives. Indeed, we would expect increased costs for blue hydrogen with a higher CO₂ capture rate, the use of zero-carbon electricity, and the procurement of low-emissions natural gas. However, a policy incentive for decarbonized hydrogen based on the reduction in life-cycle GHG emissions from gray hydrogen, such as Senator Tom Carper’s “Clean H2 Production Act,” would require blue hydrogen producers to reduce life-cycle GHG emissions below certain thresholds or forego a higher subsidy—or any subsidy at all.
Although we have focused on blue hydrogen, and particularly the GHG emissions beyond those generated at the steam methane reforming or autothermal reforming plant, concerns about life-cycle GHG emissions are not unique to blue hydrogen. The leading alternative for decarbonized hydrogen, green hydrogen, is green only if the electrolyzer uses power from zero-carbon sources. Electrolytic hydrogen using average US grid power would result in CO₂ emissions that are approximately double the CO₂ emissions from gray hydrogen. Just as green hydrogen must use zero-carbon electricity to achieve minimal emissions, blue hydrogen must procure both zero-carbon power and low-emissions natural gas to achieve a GHG footprint that’s less than 25 percent of gray hydrogen, as Figure 1 shows.
Finally, because we contend that gray hydrogen is the appropriate near-term benchmark for blue hydrogen, it is important to note that displacement of gray hydrogen is a significant opportunity for reducing US GHG emissions. 8.8 million metric tons of gray hydrogen are produced in the United States, predominantly for oil refining and ammonia production. With a CO₂ intensity of approximately 10 kilograms of CO₂ per kilogram of hydrogen, gray hydrogen accounts for nearly 90 million metric tons of CO₂ emissions— roughly1.7 percent of total US CO₂ emissions. (Of course, methane emissions add to the GHG footprint, as well.)
We cannot predict which form of decarbonized hydrogen—whether green hydrogen, low-emissions blue hydrogen, or a different production method—would be the most efficient means of displacing gray hydrogen. Establishing incentives that require life-cycle GHG emissions reductions, but do not constrain technology options, thus is the most effective policy pathway.

Read more articles from the “Potential of Hydrogen for Decarbonization” blog series:
- “Evaluating Low-Carbon “Blue” Hydrogen Against End-Use CCUS” (January 11, 2021)
- “Evaluating Zero-Carbon “Green” Hydrogen Against Renewable and Nuclear Power” (January 20, 2021)
- “Reducing Emissions in Oil Refining and Ammonia Production” (February 4, 2021)
- “Reducing Emissions in Iron and Steel Production” (February 18, 2021)
- “Effective Incentives for Hydrogen Production, with Long- and Near-Term Climate Benefits” (November 15, 2021)