Something happens at an altitude of about 60 miles: we move from "air" to "space." The exact altitude is somewhat arbitrary. It's defined roughly as where the density of the atmosphere decreases so much that a vehicle has to travel faster than orbital velocity in order to get enough lift to support itself—an aircraft becomes a spacecraft. But the point is this: mere altitude does nothing to vitiate the importance of environmental and resource economics in informing public policy on space.
On January 11, 2007, the People's Republic of China successfully destroyed one of its older weather satellites by launching a rocket that traveled at nearly 18,000 miles per hour. It hit the 6-foot long satellite, which instantly shattered into an estimated 35,000 pieces. To many experts in the national defense community, this demonstration of anti-satellite technology was neither alarming nor unprecedented. What was startling, however, was the large amount of space debris generated by the test, the largest single source since the start of the space age. But the problem was already large and growing (see Figure 1).
Space debris includes defunct spacecraft, metal shards, nuts and bolts, and a host of other discards from space activities. Debris is dangerous because it orbits at extremely high velocity; for example, mere flecks of paint have struck quarter-inch deep gouges in windows on the space shuttle. (To protect astronauts, the space shuttle has six layers of windshields.) Communications satellites, the space station, and other spacecraft have extra layers of "shielding" but still remain vulnerable to damage.
And there are no easy ways to avoid it. If a piece of debris is larger than a softball, ground-based radar can detect it and engineers can send commands to a spacecraft to maneuver it out of the way. (But this solution comes at a cost—it draws on an already limited spacecraft fuel supply.) Smaller debris is also lethal and undetectable. Debris often "begets" debris when it collides with itself to produce even more and even smaller (hence harder to detect) pieces.
To economists, space debris can be seen as one more form of pollution that can be addressed through policy mechanisms like deposit-refunds or tradable permits. For example, fees could be assessed on spacecraft at the time of launch. The size of fees can be large or small depending on the debris-generating potential of the spacecraft. (Is it painted, such that flecks of paint can become debris? Are external devices secured by lanyards? Will excess rocket propellant, which forms pellets and acts like debris, be properly managed?) Under a deposit-refund approach, any deposit payments foregone by the failure to reduce debris could accrue in a compensation fund to reimburse the cost of harm done to operating spacecraft.
Another alternative would be to issue a small number of debris permits to space-faring nations or to companies supplying launch services and spacecraft. Permits could be exchanged among low- and high-cost debris control activities associated with different types of space missions. Flexible strategies offer additional advantages. For example, some regions of space are heavily used for communications satellites or the space shuttle and space station. The stringency of strategies could be adjusted depending on the destination of the spacecraft.
Under any option, whether voluntary, mandatory, or market-like, policymakers must determine an amount of debris growth that can be appropriately managed. They must also take into account costs of complying with, monitoring, and enforcing the rules.
The Petri Dish Problem
There is an even more profound excuse for keeping space clean. One of the reasons to explore space is to pursue truly cosmic questions: Where did we come from? Are we alone? The rationale for planetary exploration is in fact to pursue these questions. But landing a robot on the surface of a planet to look for evidence of life automatically introduces our own germs into that planet's environment. The microbe we find on Mars may be our own.
Experts have long expressed concern about protecting other planets from human contamination. Safe-guards, such as assembling spacecraft in high-technology clean rooms and sterilizing all components, can be taken to minimize contamination. Using shrouds, filters, and seals can also reduce the microbes that can hitchhike from Earth Technicians might prepare inventories to document the kinds and amounts of "bio-burden" associated with spacecraft assembly and launch to provide a background record as a basis for later comparison with the spacecraft's research results.
None of these precautions fully prevents contamination. A recent report by experts engaged in the operation of rovers on Mars points out (but does not endorse) that the only way to fully eliminate risk is to stop direct-contact missions and go to passive observation." Passive observation means observing planets from a distance—say, by means of a spacecraft orbiting the planet and taking pictures. But even then, the chance remains of an accidental spacecraft crash.
Figure 1: This graphic shows the amount of spacecraft and old and new debris orbiting the Earth. Each dot represents a man-made object larger than four inches. SPACECRAFT There are more than 3,100 spacecraft orbiting Earth. Two-thirds of these are no longer active.
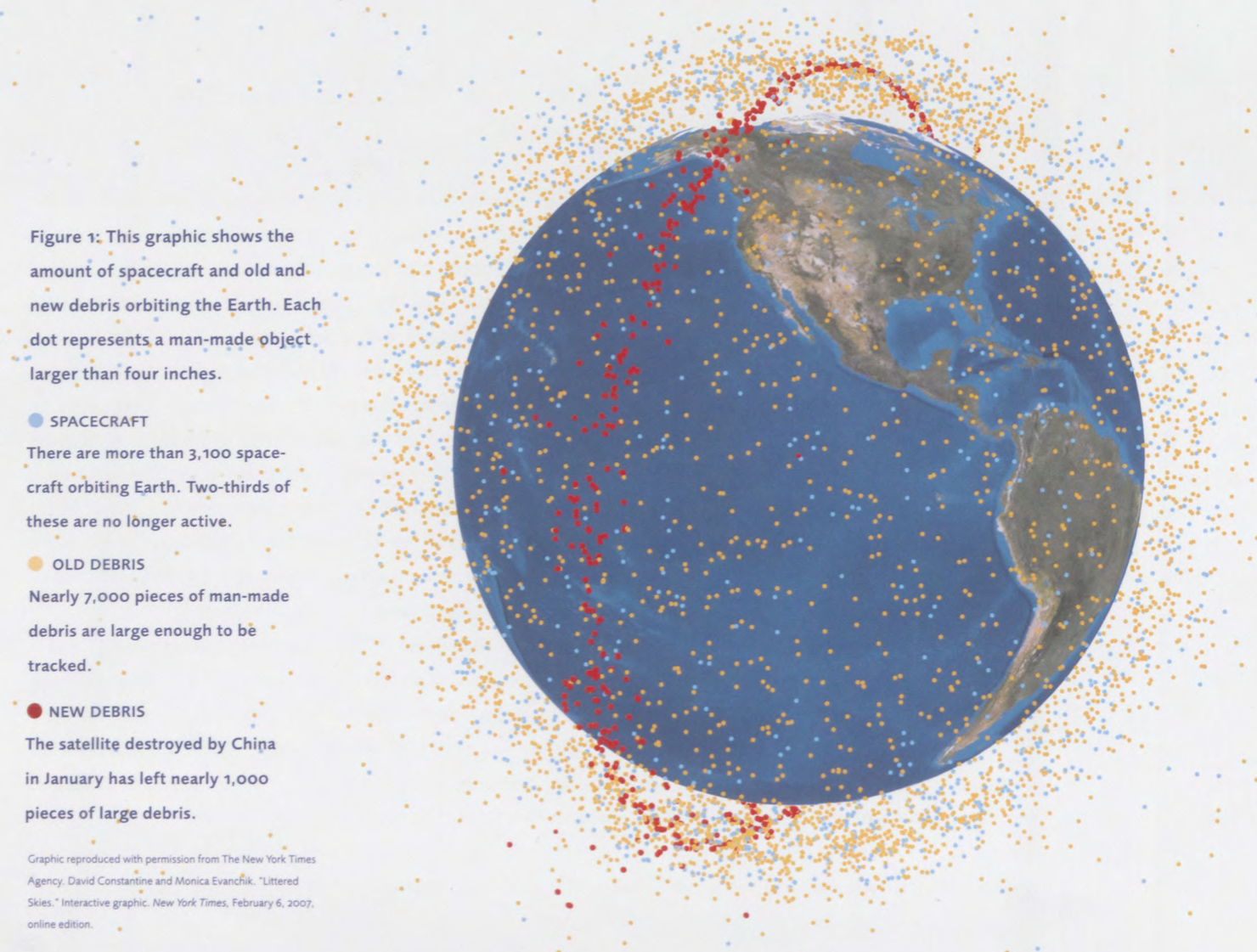
Figure 2: This figure illustrates regions and uses of the spectrum. How might planetary exploration go forward? Protecting planets and other celestial bodies while exploring space requires the balancing of competing objectives, wholly analogous to the tradeoffs involved in environmental protection on Earth.
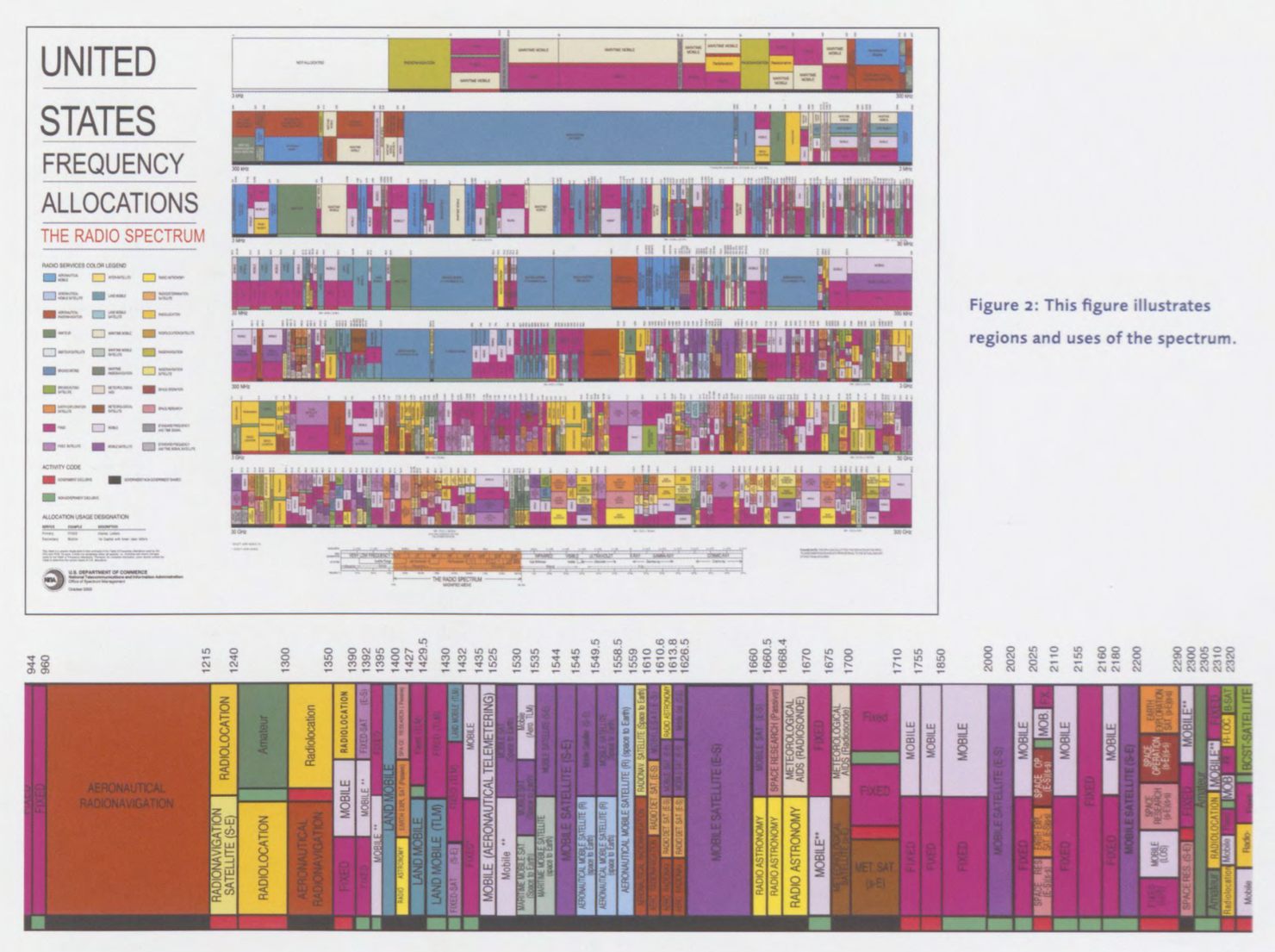
How might planetary exploration go forward? Protecting planets and other celestial bodies while exploring space requires the balancing of competing objectives, wholly analogous to the tradeoffs involved in environmental protection on Earth. An example is zoning. On some planets, researchers have identified special regions where there is a high potential for the existence of indigenous life, such as where liquid water may be present (for example, at the Martian polar caps and in areas of hydrothermal activity). International protocols now require additional sterilization requirements for spacecraft making contact these zones.
Another example of tradeoffs is figuring out how best to time the pace of exploration, given that it is frequently informed by new information about conditions for life elsewhere. The Viking landings on Mars in the 1970s suggested a dry, barren environment hostile to life. Surprisingly, recent data indicate that Mars has multiple environments, with some suitable for life. A related finding is discovery of the diversity and survivability of terrestrial microorganisms in extreme ocean environments previously deemed highly unlikely to harbor life. Given the value of this kind of information, it could be wise to adopt a "go slow" approach in space exploration, if the goal is to have as few regrets as possible about contaminating other environments.
Timing also affects the opportunity handed to future generations; those making decisions today may not be those facing consequences later. The National Research Council's Space Studies Board has urged that new efforts be directed toward research on planetary protection measures. Decisionmaking under uncertainty and discounting for intergenerational effects—long-researched topics in economics—come to the fore on a new frontier.
The National Academy of Sciences urges that scientists plan lunar and planetary studies with great care and deep concern so that initial operations do not compromise and make impossible forever after critical scientific experiments. —Preventing the Forward Contamination of Mars, Washington, DC: National Academies Press, 2005.
What's that Crosstalk?
Another emerging issue—for which the economists' toolkit has already proven useful—is allocation of a resource required for all activities in space. The electromagnetic spectrum, or airwaves, is the communications backbone, not only among everyday cell phone users and TV and radio transmission, but it is also how we communicate with space-based activities. And the airwaves are getting ever more crowded.
First, a bit of history. In 1960, University of Chicago economist Ronald Coase wrote about the difficulty of allocating the electromagnetic spectrum, responding to the problem of interference on the radio and TV dials. Coase won the Nobel Prize in Economics in 1991 partly for his argument that under certain conditions, a public good like the airwaves can be managed by conferring property rights on radio and TV stations. Rightsholders would take appropriate care to minimize mutually destructive, interfering signals. Moreover, recognizing the scarcity value of electromagnetic spectrum, they would have strong incentives to pursue innovation to develop new electronic devices capable of using it more efficiently. In 1971, RFF sponsored The Invisible Resource, a book by economist Harvey Levin that further outlined systems for and advantages of spectrum rights. By early 1995, first New Zealand, and then the United States had begun to confer property rights by auctioning portions of spectrum to the telecommunications industry.
While the auctions allocate spectrum among commercial companies, the problem has re-emerged in the case of public users. A wholly new demand for interference-free spectrum has come to the fore: satellite data to observe Earth's air, water, forests, crops, and climate. The data from these spacecraft provide early warning of changes in the Earth system, such as ice cover in the polar regions, the status of the protective ozone layer, and sea level. In some cases, experts hope that satellite data can allow monitoring of the extent of forestation, deforestation, and afforestation—measurements deemed necessary for projects such as carbon credits to facilitate forest protection by developing countries. Similarly, assessing the efficacy of the Montreal Protocol to protect the ozone layer and the effectiveness of many other international environmental agreements rely on space-derived information.
What is the connection with Coase? Every natural phenomenon, from a tree to the ocean to clouds, has a unique spectral "signature" or "fingerprint." Each emits energy (light) naturally at the microwave level or other portions of the electromagnetic spectrum. The instruments in space, then, look at Earth to detect the emitted radiation and interpret it to understand environmental parameters as varied as soil moisture, temperature, humidity, ocean salinity, and climate.
When satellites "listen" to the natural emissions, everyday electronic devices can interfere. A single cell phone uses several different transmitting devices operating at as many frequencies, substantially increasing the radio din. Figure 2 shows regions and uses of the spectrum; look hard enough to see the tiny "EEES" (earth exploration and earth science) sections where Earth "speaks" to us. The frustration cell phone callers experience with a bad signal is magnified for natural scientists trying to tune in to a highly specific natural phenomenon. In some cases, scientists aren't sure whether their signal is "real" or "noise." The National Academy of Sciences is presently carrying out a study of spectrum interference for earth science (and radio astronomy, which is also subject to the interference problem).
How best to decide who gets what? How much spectrum for our telecommunications devices, and how much to Earth science? Just as with the problems of managing space debris and planetary environments, the spectrum problem is primed for economists who have the tools with which to value public goods, frame tradeoffs among competing alternatives, and, when appropriate, suggest market-like approaches to allocating natural resources.
